Biodegradable Polymers and their Role in Drug Delivery Systems
Abstract
Biodegradable polymers are currently limited in their application in
engineering of soft tissues because they are too stiff and incompliant.
Biodegradable elastomers overcome these limitations; they have ability
to recover from multiple deformations. Citric Acid based Biodegradable
Polymers (CABEs) are a family of elastomers that have been recently
developed. Further developments in this area are still needed but they
provide
a promising new material that can be used for drug delivery systems.
Biodegradable Polymers
Biodegradable polymers are polymers that breakdown within a
limited period of time after being placed in the body and are designed
to offer temporary support. With an estimated consumption of 68
million kilograms per year in 2001 [1], biodegradable polymers
are widely used as biomedical devices and in tissue engineering
applications. Biodegradable polymers can either be natural or
synthetic. The use of synthetic polymers over naturally occurring
materials provides several advantages. Firstly, synthetic polymers
can be synthesised via a method that can be reproduced to give
the same polymer, with the exact same composition each time.
They have unlimited availability and can be produced with a wide
range of physical, chemical and mechanical properties that can be
modified depending on the application.
The most widely used synthetic biodegradable polymers
belong to the polyester family, such as Poly Lactic Acid (PLA) and
Poly Glycolic Acid (PGA) and their copolymers, such as PGLA. They
have been extensively studied and reported in the literature and
have many applications including resorbable sutures [2], surgical
fixation devices [3] and drug delivery devices. Other commonly
used biodegradable polymers include polydioxanone, which are
primarily used as a suture material, marketed as Ethicon. The
majority of the cell and drug delivery systems that have been
developed are formed using biodegradable polymers. Controlling
the release of biologically active molecules is of the uttermost
importance in tissue engineering; tissue repair and regeneration is
a complicated process and requires a large number of associated growth
factors and hormones. Thus, delivery systems that can
provide controlled release of these factors are crucial to the success
of tissue engineering [4]. Their importance is also recognised in
drug delivery systems where the development of novel systems has
been made possible largely by the advances in polymer science.
The use of biodegradable polymers offers several advantages
over other materials. Important advantages include the ability to
tailor the mechanical properties, the degradation rates and the
ability to be formed into various shapes. The main advantage of
biodegradable polymers is that their degradability reduces the need
for subsequent surgical removal, saving time and money. However,
the creation of degradation products can also be problematic.
Taylor et al. [5] reported that the degradation products of PLA
and PGA were highly toxic if they accumulated. This is because
the degradation products are acidic in nature. The result can be
a high local acid concentration, which can trigger an undesirable
local inflammatory response. This is more of a problem if a larger
implant is used, as in many orthopaedic applications [3]. They
can also be complicated and expensive to synthesise and process,
especially if further modifications are needed.
The main limitation of the commonly used biodegradable
polymers, such as α-hydroxy acid and polydioxanones, is that they
are too stiff and incompliant making them incapable of reversible
deformation. Furthermore, they do not resemble the physical
properties of human soft tissue. Their use can lead to irritation,
inflammation and scar tissue formation; consequently, preventing
integration of the device within the body. Thus, their success in
tissue engineering applications is limited [6].
Biodegradable Elastomers
This lack of suitable scaffold materials for tissue engineering
led to the development of more elastic biodegradable polymers -
biodegradable elastomers. Elastomers are polymers that exhibit
resilience and the ability to stretch and retract rapidly. Due to their
elastic nature they are able to sustain and recover from multiple
deformations without causing irritation to surrounding tissues. The
chief reason that elastomers are successful where biodegradable
polymers are not stems from their elasticity; this allows them to
recover from multiple deformations and makes them more similar
to organic human tissue. Furthermore, the mechanical properties
of elastomers can be matched to specific soft tissue structures
within the body and degradation rates can be tailored to maximise
tissue incorporation.
This improves tissue regeneration since it allows the gradual
transfer of stresses from the degrading scaffold to the newly formed
tissue. Biodegradable elastomers have been described as a “valuable
strategy for satisfying the requirements of regenerative medicine
and tissue engineering” [7]. A recently developed biodegradable
elastomer that has been successful in tissue engineering
applications is Poly Glycerol Sebacate (PGS). In 2002 Wang et al. [6]
designed, synthesised and characterised the novel biodegradable
elastomer PGS. In comparison to biodegradable polymers, PGS
demonstrates better biocompatibility; it produces significantly
less inflammation and fibrosis. This study is frequently cited which
highlights the impact of this work. PGS has since been studied
extensively and has proven to be successful in many applications,
such as drug delivery [8], supporting the growth of a variety of cells
and even wound healing [9].
Photo-Polymerisation
To realise the full capacity of a material it must be easily
processed. It is especially important to be able to use mild
techniques that will not damage molecules, particularly where the
inclusion of active molecules within the polymer are envisaged.
One alternative processing strategy that has been investigated is
photo-polymerisation. Photo-polymerisation is the use of light to
initiate crosslinking of a molecule. The main advantage of photopolymerisation
is that it allows biologically active molecules
to be incorporated within the polymer without being exposed
to harsh processing conditions because it can be carried out at
physiological temperatures. Harsh processing conditions such
as high temperatures or cytotoxic organic solvents limits the in
situ application of polymers, as well as their use as cell or drug
delivery systems. This highlights the large, unmet need for milder,
gentler processing methods; a problem which can be overcome by
using photo-polymerisation. Furthermore, photo-polymerisation
can occur directly in or on the tissue, providing the advantage
of localised delivery, ease of application and reduction in dosage
amount if used as a delivery vehicle.
Hubbell et al. were the first to demonstrate in vivo
photopolymerisation of degradable polymers. They successfully
demonstrated that it could be used for the prevention of postoperative
adhesions. Further examples include: Hubbell et al. [10]
who investigated PEG-oligoglycolyl-acrylates as a controlled release
carrier and Nivasu et al. [11] who looked at controlled release of the
antibiotic sulfamethoxazole.
Citric Acid Based Biodegradable Polymers (CABEs)
Citric acid based biodegradable polymers CABEs are a family of
polyester elastomers that have been recently developed. Poly(diol
citrates) were the first group of CABEs to be synthesised and
have been most widely studied. Synthesis of poly(diol citrates) is
based on polymerisation of a linear diol with citric acid (Figure 1).
Citric acid (Figure 2) is a multifunctional molecule which provides
pendant functionality and is a non-toxic by-product of the Krebs
cycle. It is FDA approved and currently used in clinical settings
as an anticoagulant [12]. The primary advantage of CABEs over
other polymers is their ease of synthesis. They are formed via
poly-condensation reactions without the use of toxic catalysts
or crosslinking reagents [13]. The monomers are also readily
available, relatively inexpensive and importantly non-toxic, even
once they have been degraded. They are also synthesised in very
mild conditions in a short space of time. CABEs can be synthesised
at temperatures as low as 135oC in a reaction taking only 125
minutes, whereas many of the biodegradable polymers reported in
the literature have very complex and costly synthesis procedures.
For example, the synthesis of Polyhydroxyalkanoates (PHAs) [14]
has a very complicated fermentation synthesis procedure and
the reactions to form polyesters such as PGLA can take up to six
hours and requires temperatures of up to 200oC. Furthermore,
the formation of elastomers from polymers of D,L-lactide [15] and
the synthesis reaction for PGS can both take up to three days to
complete [13].
Figure 1: Reaction for Poly(diol citrate) synthesis.
The poly-condensation reaction produces a crosslinked
polyester network with degradable ester bonds [16] and the elastic
nature of the molecule is conferred by this crosslinked network.
The reaction preserves the pendant functionality of the citric
acid molecule for potential conjugation with biological molecules
without the need for further modification. This is because citric
acid is a multifunctional molecule; it removes the need for further
modification of the polymer, saving time and money. The low
cost of the monomers and the simple synthesis procedure of
CABEs increases their potential for commercialisation. This is an
important consideration that can affect the clinical implementation
of materials. One of the most unique aspects of CABEs is ease with
which their physical properties can be controlled. These properties
have been shown to be controllable by
i) Changing the diol used [13]
ii) Changing the molar ratio of the monomers [17],
iii) Changing the synthesis conditions, including temperature
[17] and
iv) Changing the polymerisation conditions [7]. Modification
of these conditions can also modulate degradation rates [13];
this allows CABEs to be tailored to specific applications unlike
other biodegradable polymers.
Development of CABEs
The first CABE was synthesised by Yang et al. [13]. Citric acid
was reacted with a range of diols, including 1,8-Octanediol, to create
the novel Poly(1,8-Octanediol- Co-Citric Acid) (POC) biodegradable
elastomer. POC demonstrates good mechanical properties with an
ultimate tensile strength as high as 6.1MPa and Youngs modulus
with a range of 0.92-16.4 MPa, making it within the range of
many soft tissues in the body. Yang also demonstrated that POC
had excellent biocompatibility; it supports the attachment and
proliferation of Human Aortic Smooth Cells (HASMC) and Human
Aortic Endothelial Cells (HAEC). The cells were able to attach to the
surface without needing any pre-treatment or surface modification.
POC also demonstrated an in vivo inflammatory response similar
to PLGA, a degradable polymer widely accepted as biocompatible
[14-17].
Since their initial development, they have been extensively
studied and further research has led to a number of new key
developments Owing to their unique properties CABEs have been
utilised in a wide variety of applications; the most successful being
cardiovascular tissue engineering. Yang et al. developed a biphasic
tubular POC scaffold for use as small diameter blood vessel grafts.
The mechanical properties of the scaffold are very similar to
properties of native human arteries and veins. In addition, Kibbe et
al. [18] provides evidence for POC as a non-thrombogenic coating
for blood contacting devices. Kibbe et al. demonstrated that when
current ePTFE grafts are coated in POC it resulted in improved
endothelial cell adhesion and reduced platelet adhesion compared
to standard ePTFE grafts. Since their initial development their
repertoire has greatly expanded due to further research in the
area owing to the promising results initial studies into CABEs have
produced.
Drug Delivery Systems (DDS)
Drug delivery is a fast growing field with an unmet need for
more effective materials for drug delivery. Drug delivery systems
overcome the limitations imposed by conventional delivery routes
such as oral, intravenous and intramuscular, which prevent effective
or efficient drug delivery. Conventional delivery routes typically release
a large amount of the drug initially, over a very short period of
time (Figure 3). There is then a decline in the plasma concentration
of the drug and administration of another dose becomes necessary
to return the plasma concentration of the drug to therapeutic levels.
This results in multiple administrations of the drug since the therapeutic
dose cannot be sustained (Figure 4).
Figure 3: Conventional drug release profiles for intravenous,
intramuscular and oral administration.
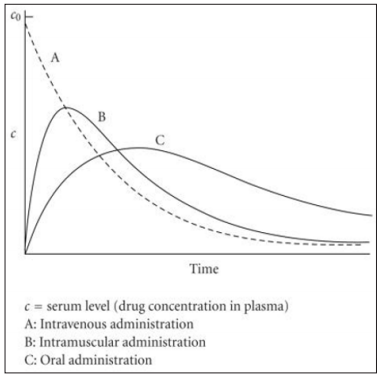
This can be difficult for patients to maintain and has several
disadvantages. Firstly, patients need to have frequent repeat doses
of the drug which can be inconvenient and time consuming, resulting
in reduced patient compliance. Furthermore, a large dose of the
drug has to be administered because by the time it reaches the site
of action only a small percentage of the initially administered dose
remains. Consequently, a high concentration of the drug remains in
the systemic circulation and, depending on the drug, this can cause
a variety of unwanted side effects. This is particularly important if
the drug is highly toxic, such as chemo-therapeutic drugs. These
conventional delivery methods are seen as inadequate for the
delivery of many therapeutic agents, especially those that have very
short half-lives, such as peptides, genes and recombinant proteins,
and would therefore need to be administered very frequently to
maintain a therapeutic dose.
Drug delivery systems provide a solution for more effective
drug delivery. Gregoradis first proposed the use of carriers to
deliver and release drugs in a controlled manner at a specific site
in the 1970’s. A primary advantage of drug delivery systems is that
they allow controlled and sustained release of the drug (Figure 5).
This essentially eliminates the need for patients to take multiple
doses of a drug and can therefore improve patient compliance
and convenience, particularly for drugs that require frequent
administration. The drug can also be delivered locally, close to its
site of action. This reduces the amount of drug that is needed and
minimises the systemic concentration of the drug, consequently
reducing the likelihood of side effects.
Polymeric Drug Delivery Systems
Polymer science has been the backbone of the development
of new drug delivery systems for the past few decades. Polymeric
materials are widely used in drug delivery because they have been
extensively studied and shown to be biocompatible, non-toxic
and have tunable mechanical properties and degradation rates.
The polymeric system can also provide a form of protection for
the drug, preventing exposure to the physiological environment.
Therefore, improving the stability of the drug and increasing its
bio-availability. Hence the choice of polymer is one of the most
important considerations.
One example of a successful polymeric drug delivery system
is Norplant, an implantable contraceptive device. It was oneof
the first drug delivery systems to be widely used in clinical
practice. However, it is a non-degradable system and therefore
requires surgical removal once the drug has depleted. Its clinical
use has declined, and it has been discontinued in the UK and US.
This is largely attributed to the problems of removal, including
the possibility of complications during the retrieval process, the
risk of infection and lack of patient compliance [19]. The use of
biodegradable polymers presents a far more attractive option as
there is no need for surgical removal once the drug has depleted.
Many biodegradable polymers have been investigated for use
in drug delivery and have established a role in controlled drug
release. Aliphatic polyesters such as poly(lactic acid), poly(glycolic
acid), poly(lactide-co-glycolide), poly(decalactone) and poly(εcaprolactone)
have been the subject of the most extensive
investigations [20]. Many biodegradable polymers have been
successfully fabricated into a number of devices for drug delivery
including microspheres, microcapsules and nanoparticles [21,22].
There are several ways that polymers can be utilised in drug
delivery, including diffusion controlled systems, swelling controlled
devices and particulate systems such as polymer-drug conjugates.
This study will look at chemically controlled delivery systems,
where the drug will be dispersed in a biodegradable polymeric
matrix and released upon degradation of the cross-linked network
(Figure 6).
Figure 6: Conventional drug release profiles for intravenous,
intramuscular and oral administration showing
repeated dose administration ( indicated by arrows) over
time.
Although many new delivery methods have been developed,
very few actually go on to be implemented in clinical practice. This
is partly due to the problems with production. If a system is to
be implemented on a large scale it must be easily produced; this
is where CABEs could be successful over other delivery methods.
One important problem with other biodegradable drug delivery
systems it that that they often require several processing steps
after drug entrapment before they can be used. In contrast, CABEs
require very little processing after drug entrapment and can trap
drugs under physiological conditions. This step can be a cause of
drug deactivation in other systems.
There is currently only one study that has reported the use of
CABEs in drug delivery. Hoshi et al. [23] used nanoporous Poly(1,8-
Octanediol-Co-Citrate) (POC) to form a drug delivery system to
entrap Dextran, an anti-platelet drug. The results showed that POC could
release Dextran at a slow, controlled rate and therefore could
potentially be used in drug delivery applications. In addition to this,
previous studies evaluating the properties of CABEs [13,24] have
reported that they have the potential for successful application
in drug delivery but have not carried out any controlled release
studies.
In Situ Drug Delivery Systems
Although in situ polymerisation has its advantages, the
conditions required are very specific. The physiologically acceptable
temperatures are within a very narrow range and the system must
be rapidly polymerised if it is to be successfully implanted in a
clinical setting [25]. The advantage of photo-initiated crosslinking
is that the reaction proceeds rapidly at low temperatures and
therefore may be suitable for the incorporation of thermally
sensitive drugs such as peptides and proteins. Photopolymerisable
systems have an advantage over other types of in situ systems, such
as chemically initiated systems. This was demonstrated by Dunn
et al. [26] who crosslinked biodegradable co-polymers D,L lactide
and L-lactide with ε-caprolactone, using a chemically initiated
thermo-set system, for use as a slow-release drug delivery system.
Disadvantages of the system include taking up to 30 minutes to set
and the highly exothermic nature of the crosslinking reaction could
result in tissue necrosis. There was also burst release of the drug in
the first hour which could result in the appearance of side effects
[27-40].
Drug delivery systems that can be formed in situ have
evolved from the need for prolonged and better control of drug
administration. They offer a wide range of advantages over other
drug delivery systems. Firstly, the drugs can be administered
very easily by injecting into the desired site, making it quick and
relatively painless for the patient, which can increase patient
compliance. They also allow localised and sustained drug release
over a prolonged period of time. Furthermore, it can be moulded into
any desired shape, regardless of complexity, which is very difficult
to achieve with other methods of drug delivery [20]. For example,
PGLA microspheres are very difficult to administer because they
require a surgical incision to gain access to the desired site. They
also require prior preparation before they can be injected into the
body, making it inconvenient for the patient [21]. In situ systems
are relatively easy to synthesise, making them excellent candidates
for clinical use.
Conclusion
Although many advances in polymers and drug delivery
systems and methods have been made over the years, there is
still a need for new, unique materials owing to the advances in
pharmaceuticals. There is also a need for more gentile and facile
methods of biological molecule inclusion. Photo-polymerisation
provides an effective solution. Further research into this area could
lead to the development of the optimal drug delivery system.
The Role of Carvacrol as Active Compound
of Essential Oils in Diabetes - https://biomedres01.blogspot.com/2020/03/the-role-of-carvacrol-as-active.html
No comments:
Post a Comment
Note: Only a member of this blog may post a comment.